Muscle Contraction
Table of Contents
What is a Muscle Contraction?
The activation of tension-generating areas inside muscle cells causes contractions in the muscles. In physiology, a muscular contraction does not always imply a shortening of the muscle since stress can be created in the muscle without a change in length, as in the case of keeping a heavy object in place.
muscular relaxation, or the return of the muscular fiber to its low tension-generating condition, occurs after the cessation of muscle contraction.
The interaction of two types of filaments—thin and thick filaments—is essential for the muscle cells to produce contractions.
Actin strands coiled around one another to create a chain are the primary component of thin filaments, whereas motor protein muscle chains predominate in thick ones.
These two filaments come together to produce myofibrils, which are fundamentally useful organelles in the skeletal muscle system.
Skeletal muscle contractions in vertebrates are neurogenic because they depend on motor neurons’ sensory input. Multiple muscle fibers can be innervated by a single motor neuron, which results in the contraction of the fibers simultaneously.
The sliding filament theory explains how once innervated, the protein filaments within each skeletal muscle fiber move past one another to create a contraction.
Depending on the action potential frequency, the resulting contraction can be characterized as a twitch, summation, or tetanus.
The length-tension connection states that when skeletal muscles are stretched to an intermediate length, the muscle’s tension reaches its maximum.
Although they can be influenced by autonomic nervous system stimulation, the contractions of smooth and cardiac muscles, opposite to the contractions of skeletal muscle, are myogenic (i.e., they are started by the smooth or heart muscle cells themselves after being stimulated by external events like nerve stimulation). These muscle tissues’ methods of contraction are comparable to those of skeletal muscle tissues.
The two additional factors that may be used to define muscle contraction are length and tension.
Muscle contractions are complex in spontaneous motions that underpin locomotor activity because they can result in time-varying variations in length and tension.
Therefore, in skeletal muscles that contract during movement, neither length nor tension are expected to be constant.
If the muscle length is constant but the muscular tension varies, the contraction is said to be isometric. On the other hand, if muscular tension doesn’t change during a contraction, the muscle contraction is said to be isotonic. When a muscle shortens, a concentric contraction occurs. An eccentric contraction occurs when the length of the muscle lengthens.
Types of Muscle Contraction
The two parameters of force and length can be used to characterize muscle contractions. One can distinguish between two types of forces: tension and load.
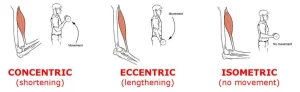
The weight is the force that an item applies to a muscle, whereas muscular tension is the force that a muscle applies to an object.
An isometric contraction occurs when there are changes in muscular tension but no corresponding changes in muscle length.
An isotonic muscular contraction occurs when there is a change in muscle length but not in muscle tension.
Muscle length can either lengthen to create an irregular contraction in an isotonic contraction or shorten to produce a concentric contraction.
Muscle contractions are complex in spontaneous motions that support movement because they can result in time-varying differences in length and tension.
Therefore, while the muscle is working during locomotor activity, neither length nor tension is likely to remain constant.
Isometric contraction
A muscle that is contracted isometrically produces tension without altering the length.
An illustration of this is when the hand and forearm muscles grasp an object; the hand joints remain immobile, but the muscles produce enough force to keep the thing from falling.
Used In:
- Standing
- Sitting
- Postural Control
Isotonic contraction
When a muscle contracts isotonically, its length changes but its tension stays the same. When the force of contraction of a muscle equals the entire load on the muscle, this happens.
Most Of The Time Movement Is Of This Types
Used In:
- Walking
- Running
- Movement Of a part of the body ( eg. Hand Movement)
Concentric contraction
When a muscle contracts concentrically, its tension is enough to resist the load, and the muscle gets shorter during the contraction.
This happens when the load resisting the muscle’s contraction is greater than the force the muscle generates. The sliding filament theory states that a muscle is prompted to contract during a concentric contraction.
This happens down the muscle, producing force at the insertion and origin, shortening the muscle, and altering the joint’s angle.
When the hand went from the knee to the shoulder, a concentric contraction of the biceps would force the arm to bend at the elbow (a biceps curl). The angle of the joint in the triceps would alter with a concentric contraction.
The arm would straighten and the hand would move towards the leg with a concentric contraction of the triceps changing the joint’s angle in the opposite direction.
Eccentric contraction
When a muscle contracts differently, its muscular fibers stretch because the tension created during an isometric contraction is not enough to counteract the external load on the muscle.
The muscle operates to decelerate the joint after a movement or to regulate the repositioning of a load, instead of pulling the joint in the direction of the muscular contraction. This may happen willingly or involuntarily.
Muscular strength appears to be increased more quickly by strength training that combines eccentric and concentric contractions than by training alone with concentric contractions. However, longer contractions also result in more muscle damage from training.
The elbow bends during the beginning of an eccentric contraction of the biceps muscle, then straightens as the hand travels away from the shoulder. The elbow begins the movement straight during an eccentric contraction of the triceps muscle and bends as the hand approaches the shoulder.
In contrast to cross-bridge cycling in concentric contractions, the mechanism behind the involvement of desmin, titin, and other z-line proteins in eccentric contractions is poorly known.
Chemical energy is still used by the muscle even though it is not producing as much mechanical effort as it would in a concentric contraction with the same power. walking up a flight of stairs, for instance, requires more energy than walking down the same level.
When overworked, muscles under severe eccentric loading sustain more damage than those under concentric stress.
Eccentric contractions are typically referred to as negatives when they are utilized in weight training. The Z-lines are drawn together by the sliding of myosin and actin contractile muscle myofilaments past one another during a concentric contraction.
The myofilaments slide past one another in the opposite direction during an eccentric contraction, yet it is unknown how the myosin heads move during this process.
Workouts with a high eccentric load can sustain a higher weight, but they also cause more muscle damage and pain that doesn’t start for one to two days after training. Strength increases can be higher with exercises that include eccentric and concentric muscle contractions than with only concentric contractions.
Eccentric contractions in movement
To prevent injury to joints, eccentric contractions often happen as a braking force against concentric contractions. Eccentric contractions help to maintain smooth motions during almost any normal activity, but they can also slow down quick movements like a punch or throw. Reducing eccentric braking during fast motion training, like pitching in baseball, enables more power to be created throughout the movement.
Research is being done to determine whether eccentric contractions might expedite the healing process for weakened or damaged tendons. High-load eccentric contractions have been demonstrated to be beneficial for treating patellar tendinitis and Achilles tendinitis.
Vertebrate
Skeletal, smooth, and cardiac muscle tissues are found in vertebrate animals. Most of the body’s muscular mass is made up of skeletal muscle, which is what drives movement. The gastrointestinal system, blood arteries, and other parts of the body that undergo prolonged contractions are formed by smooth muscle.
The heart’s cardiac muscle is responsible for pumping blood. The highly ordered alternating arrangement of A bands and I bands in skeletal and cardiac muscles gives them a striped look under a microscope, thus the term “striated muscle.”
Skeletal muscle
All contractions of skeletal muscles, except reflexes, are brought about by impulses that start in the brain. The motor neuron that innervates several muscle fibers receives electrical signals from the brain via the neurological system.
Certain reflexes have a feedback loop with the grey matter in the spinal cord that allows the signal to contract to come from there. There is a reflex component to other movements like breathing, eating, and moving; the contractions might be started intentionally or involuntarily.
Neuromuscular junction
A chemical connection created when a motor neuron and a muscle fiber come into contact is known as a neuromuscular junction. It serves as the location where a motor neuron sends a signal to a muscle fiber to start a contraction.
An action potential is first generated in the motor neuron’s cell body, and it is then transmitted via saltatory conduction up its axon towards the neuromuscular junction, where it causes the depolarization of the muscle fiber at the neuromuscular junction.
The voltage-gated calcium channels allow the action potential to enter the terminal bouton and initiate a Ca2+ ion flow.
Acetylcholine is released into the synaptic cleft that separates the motor neuron terminal from the neuromuscular junction of the skeletal muscle fiber as a result of the Ca2+influx causing synaptic vesicles containing the neurotransmitter to fuse with the plasma membrane.
Nicotinic acetylcholine receptors on the neuromuscular junction are bound by acetylcholine, which then diffuses across the synapse and activates them.
When the intrinsic sodium/potassium channel of the nicotinic receptor is opened, potassium leaks out and sodium rushes in. As a result, when sodium enters, the sarcolemma fast changes polarity and increases in voltage from its resting membrane potential of -90 mV to as high as +75 mV.
After potassium leaves, the membrane potential becomes hyperpolarized and is subsequently restored to its resting state. The end-plate potential is the name given to this abrupt variation.
Excitation–contraction coupling
The mechanism by which a muscle action potential in the muscle fiber induces myofibrils to contract is excitation–contraction coupling (ECC). Excitation-contraction coupling in skeletal muscles is dependent on a direct coupling between two essential proteins: the voltage-gated L-type calcium channel known as dihydropyridine receptors (DHPRs) and the sarcoplasmic reticulum (SR) calcium release channel known as ryanodine receptor 1 (RYR1).
The RyRs live across the SR membrane, but the DHPRs are found on the sarcolemma, which includes the transverse tubules and the surface sarcolemma. Known as a trio, excitation–contraction coupling mostly occurs near a transverse tubule and two SR areas containing RyRs.
Excitation-contraction coupling (ECC) happens when a muscular action potential is produced by the depolarization of skeletal muscles, which often happens as a result of neuronal innervation.
Depolarizing the inner part of the muscle fiber, this action potential travels from the muscle’s surface into the network of T-tubules within the muscle fiber. This triggers the activation of ryanodine receptors in the nearby sarcoplasmic reticulum via dihydropyridine receptors in the terminal cisternae.
Through foot mechanisms, which involve conformational changes that allosterically activate the ryanodine receptors, the activated dihydropyridine receptors physically engage with the ryanodine receptors to activate them.
A calcium flash occurs when ryanodine receptors activate, releasing Ca2+ from the sarcoplasmic reticulum into the immediate junctional space and allowing it to diffuse into the bulk cytoplasm.
The action potential increases calcium levels throughout the cell and triggers the almost simultaneous activation of hundreds of calcium sparks, which results in the upstroke of the calcium transient.
The actin filaments link Troponin C to the Ca2+ that is released into the cytoplasm. Actin filaments can execute cross-bridge cycling thanks to this link, which can result in force and, in certain cases, motion.
There are several rapid routes to relaxation once the required motion is completed. A Ca2+ buffer that has different cytoplasmic proteins binding to Ca2+ with extremely high affinity can rapidly induce relaxation.
These proteins found in the cytoplasm enable rapid twitch muscles to relax quickly. Until the next action potential occurs, a persistent relaxation is produced by the active pumping of Ca2+ back into the sarcoplasmic reticulum by the Sarco/endoplasmic reticulum calcium-ATPase (SERCA), although at a reduced rate.
In addition, mitochondria take part in the absorption of Ca2+, which they then transfer to SERCA where it is stored in the sarcoplasmic reticulum. The total removal of Ca2+ from the cells is also accomplished via a few relaxation mechanisms, including NCX, Ca2+ pumps, and Ca2+ leak channels.
Ca2+ is released from Troponin C as the concentration of Ca2+ drops to resting levels, preventing cross-bridge cycling and resulting in a decrease in force and relaxation. The muscle can contract once more after complete relaxation, completely restarting the cycle.
Sliding filament theory
The mechanism by which muscles contract is explained by the sliding filament theory. Tension in the muscle is produced by a thin filament sliding across a thick filament as a result of a series of repeated actions. Hugh Huxley and Jean Hanson created it independently in 1954, as did Andrew Huxley and Rolf Niedergerke.
According to biology, the sarcomere’s contraction is not uniform throughout; during contraction, the thick filaments’ central location becomes unstable and may shift, but the titin-elastic myofilament acts to counteract this. By drawing the thick filament into the center, this tiny myofilament keeps the sarcomere’s tension constant.
Cross-bridge cycle
The sliding filament idea is based on a series of chemical processes known as cross-bridge cycling. A cross-bridge is a protrusion of myosin made up of two heads that protrude from thick filaments.
Two binding sites exist on each myosin head: one for actin and the other for adenosine triphosphate (ATP). Myosin can attach to another actin molecule when ATP is bound to a myosin head, separating myosin from actin.
Myosin uses the energy it releases from hydrolyzing the ATP after it is engaged to shift into the “cocked position,” where it binds weakly to a portion of the actin-binding site. Tropomyosin blocks the rest of the actin-binding site. Adenosine diphosphate (ADP) + Pi are now present in the tilted myosin head due to the hydrolysis of ATP.
Actin filaments’ troponin C binds to two Ca2+ ions. Tropomyosin slides across and unblocks the remaining portion of the actin-binding site as a result of the troponin-Ca2+ combination.
The two myosin heads close and myosin forms a tight bond with actin when the remaining actin binding sites are unblocked. After releasing the inorganic phosphate, the myosin head starts a power stroke that produces a force of two pN.
The sarcomere is shortened by the power stroke, which causes the actin filament to migrate inward. After that, myosin releases ADP while still being firmly attached to actin. Myosin remains linked to actin in a rigid form until additional ATP attaches to it after the power stroke when ADP is released from the myosin head.
The stiffness condition that is indicative of rigor mortis would be brought on by a deficiency of ATP. The myosin head will separate from actin once more once another ATP attaches to it, initiating a new cross-bridge cycle.
For as long as the cytoplasm contains enough ATP and Ca2+, cross-bridge cycling may proceed. When Ca2 is aggressively pushed back into the sarcoplasmic reticulum, cross-bridge cycling may end.
Tropomyosin reverts to its initial conformation to obstruct the binding sites once again after Ca2+ is removed from the thin filament. The muscle relaxes when the myosin stops attaching to the thin filament.
To keep the Ca2+ion concentration in the sarcoplasm constant, the Ca2+ions depart the troponin molecule. The fluid surrounding the myofibrils becomes deficient as a result of the vigorous pumping of Ca2+ ions into the sarcoplasmic reticulum.
As a result, the troponin loses its Ca2+ ions. As a result, contraction stops when the tropomyosin-troponin complex once more covers the actin filament binding sites.
Gradation of Skeletal Muscle Contractions
Sweat, summation, and tetanus are three main categories into which the power of skeletal muscular contractions can be divided. A twitch is a single action potential generated by the muscle fiber itself that consists of a contraction and relaxation cycle.
The latent period, which typically lasts 10 ms, is the interval between a motor nerve stimulus and the innervated muscle contracting as a result.
It is caused by the length of time it takes for the nerve action potential to propagate, the chemical transmission time at the neuromuscular junction, and the subsequent steps in the excitation-contraction coupling.
A summation would result from a muscle twitch that simply sums onto the preceding twitch if another muscular action potential were to be generated before the muscle twitch fully relaxes.
There are two methods for achieving summing: multiple fiber summation and frequency summation. By altering the frequency at which action potentials are sent to muscle fibers, frequency summation regulates the force produced by the skeletal muscle.
A portion of the muscle’s fibers will fire at any moment during a contraction because action potentials do not reach muscles simultaneously.
About one-third of the fibers in each muscle will fire at once under normal circumstances when people are using their muscles as hard as they can consciously contract them[citation needed].
However, this ratio can vary depending on several physiological and psychological factors (such as Renshaw cells and Golgi tendon organs). To prevent tendon avulsion, the ‘low’ degree of contraction serves as a protective mechanism.
A 95% contraction of the fibers generates enough force to harm the body. When there is multiple fiber summation, the smaller motor units are activated before the bigger ones because they are more excitable when the central nervous system delivers a weak signal to contract a muscle.
larger motor units can have up to 50 times the contractile power of smaller motor units, and as the signal strength increases, both bigger and smaller motor units are activated. The power of muscle contraction increases with the number and size of motor units that are engaged.
The gradation of muscular force during mild contraction can occur in tiny increments, which then grow progressively bigger when greater amounts of power are required. This phenomenon is known as the size principle.
Lastly, a muscular contraction is considered tetanus if the frequency of muscle action potentials rises to the point where the muscle contraction reaches its maximum force and plateaus at that point.
Length-tension relationship
The length of the muscle at which an isometric contraction occurs and its strength are related by the length-tension connection. When muscles are near their optimum length, which is usually their resting length, they contract with the highest active tension.
The maximum active tension produced diminishes as the muscle is stretched or shortened beyond this point (either by the muscle’s activity or by an external force).]For modest deviations, this decrease is negligible, but as the length becomes farther from the ideal, the tension rapidly decreases.
Because muscle cells include elastic proteins like titin and extracellular matrix when a muscle is stretched over a certain length, a completely passive tension develops that inhibits the muscle’s ability to extend.
Together, these factors make it difficult to extend an active muscle over the point of maximum active tension.
Force-velocity relationships
The force-velocity connection connects the rate of muscle length change—which is often controlled by outside factors like load or the force of other muscles—to the force that the muscle produces.
As the shortening velocity grows, the force decreases in a hyperbolic manner about the isometric force, ultimately approaching zero at a maximum velocity. When a muscle is stretched, on the other hand, the force grows above its isometric maximum until it ultimately reaches its absolute maximum.
The active damping of joints actuated by opposing muscles that are also active at the same time is facilitated by this intrinsic feature of active muscle tissue. In these situations, the force-velocity profile increases the lengthening muscle’s force production at the shortening muscle’s expense.
This preference for the muscle that brings the joint back into balance improves the dampening of the joint. Furthermore, when muscular force grows, so does the damping’s strength. Thus, by simultaneously contracting opposing muscle groups (co-contraction), the motor system may actively regulate joint damping.
Smooth muscle
The two subgroups of smooth muscles are single-unit and multi-unit. The gastrointestinal tract and blood arteries contain single-unit smooth muscle cells.
These cells can contract as a functioning syncytium because gap junctions connect them. Smooth muscle cells exhibit myogenetic contraction, subject to modulation by the autonomic nervous system.
Multiunit smooth muscle cells are located near the base of hair follicles and the muscle of the eye, in contrast to single-unit smooth muscle cells.
Autonomic nervous system neurons independently trigger the contraction of multiunit smooth muscle cells. As so, they permit progressive responses and fine control, akin to the recruitment of motor units in skeletal muscle.
Mechanisms of smooth muscle contraction
Multiple inputs, including spontaneous electrical activity, neurological and hormonal inputs, local changes in chemical composition, and strain, can alter the contractile activity of smooth muscle cells, which can be either tonic (sustained) or phasic (transient).In contrast, skeletal muscle cells’ contractile action depends on a single brain input.
Action potentials, which often follow a slow wave potential or pacemaker potential, can be spontaneously generated by some kinds of smooth muscle cells. Rather than Na+, the influx of extracellular Ca2+ is what causes these action potentials. Cytosolic Ca2+ ions are necessary for crossbridge cycling in smooth muscle cells, much like in skeletal muscles.
The extracellular Ca2+ that enters through calcium channels and the Ca2+ ions that are released from the sarcoplasmic reticulum are the two sources of cytosolic Ca2+ in smooth muscle cells. Myosin light-chain kinase is bound and activated by calmodulin, which is bound by increased cytosolic Ca2+.
Myosin is phosphorylated on the 20 kilodaltons (kDa) myosin light chains on amino acid residue-serine 19 by the calcium-calmodulin-myosin light-chain kinase complex. This phosphorylation facilitates the molecular contact between myosin and actin, starts contraction, and activates the myosin ATPase. Smooth muscle cells do not have troponin, in contrast to skeletal muscle cells, however, they do have the thin filament protein tropomyosin and two other important proteins, caldesmon and calponin.
Therefore, unlike in skeletal and cardiac muscles, smooth muscle contractions are brought on by Ca2+-activated phosphorylation of myosin rather than Ca2+ binding to the troponin complex, which controls myosin binding sites on actin.
Myosin light chain phosphatase eliminates the phosphate groups from the myosin heads, ending cross-bridge cycling and putting the muscle in a latch state. Smooth muscle contraction velocity is positively correlated with the phosphorylation of the 20 kDa myosin light chains.
As indicated by oxygen consumption, there is a sharp spike in energy demand at this time. Force in tonic smooth muscle is maintained even after a few minutes of commencement, when the calcium level, phosphorylation of the 20 kDa myosin light chains, and energy consumption all significantly drop.
Force is produced during muscle contraction by quickly cycling cross-bridges between phosphorylated myosin and activated actin. Dephosphorylated “latch-bridges” are thought to be the cause of force maintenance, as they slowly cycle and sustain force.
It is thought that many kinases, including protein kinase C, DAPK3, and rho kinase, are involved in the prolonged period of contraction, and Ca2+ flow may be important.
Neuromodulation
The autonomic nervous system can control the rate and intensity of smooth muscle contractions, even though they are myogenic. Acetylcholine is a neurotransmitter released by the parasympathetic nervous system‘s postganglionic nerve fibers. It binds to smooth muscle cells’ muscarinic acetylcholine receptors (mAChRs).
These receptors are G-protein coupled, or metabotropic, meaning they start a second messenger cascade. In contrast, the neurotransmitters norepinephrine and epinephrine are released by the sympathetic nervous system’s postganglionic nerve fibers and attach to metabotropic adrenergic receptors.
The particular properties of the activated receptor determine the precise actions of the smooth muscle; both sympathetic and parasympathetic input can be either excitatory (contractile) or inhibitory (relaxing).
Cardiac muscle
Heart muscle cells come in two varieties: contractile and autorhythmic. Instead of contracting, autorhythmic cells set the tempo at which other cardiac muscle cells contract; this contraction is controlled by the autonomic nervous system. On the other hand, the bulk of the heart muscle is made up of contractile muscle cells, or cardiomyocytes.
Excitation-contraction coupling
Depolarization conduction and Ca2+ release mechanisms happen in the excitation-contraction (E-C) coupling of both skeletal and cardiac muscle. Despite their similarities, the proteins in question have different structures and control modes. multiple genes encode the dihydropyridine receptors (DHPRs), whereas multiple isoforms of the ryanodine receptors (RyRs) exist. Additionally, the L-type calcium channel (DHPR on cardiac myocytes) and RyR2 (the main RyR isoform in cardiac muscle) are not physically coupled in cardiac muscle; instead, they are faced with a junctional coupling. This means that DHPR contacts with RyR1 (the main RyR isoform in skeletal muscle) to regulate Ca2+ release in skeletal muscle.
In contrast to skeletal muscle, it is believed that the primary mechanism responsible for E-C coupling in cardiac muscle is calcium-induced calcium release. This process is based on the junctional structure that exists between the T-tubule and sarcoplasmic reticulum. Both the integrity of the T-tubule and its structure depend on junctophilin-2 (JPH2).
Receptor accessory protein 5 (REEP5) is another protein that maintains the typical shape of junctional SR. Deficiencies in any of the two proteins can lead to defects in junctional connections. The passage of Ca2+ via the L-type calcium channels acts as a calcium trigger, activating RyR2s during the calcium-induced calcium release mechanism. Following this, diad formations rather than triads are more common in heart muscle.
When an action potential is started by pacemaker cells in the atrioventricular or sinoatrial node and transmitted to every cell in the heart via gap junctions, this is known as an excitation-contraction coupling in cardiac muscle cells.
The action potential travels along the surface membrane into T-tubules (these are not present in all cardiac cell types), and during the early portion of the plateau phase, the depolarization causes extracellular Ca2+ to enter the cell through L-type calcium channels and possibly the sodium-calcium exchanger (NCX).
Despite making up just 10% of the Ca2+ required for activation, this Ca2+ influx is comparatively greater than that of skeletal muscle. Intracellular Ca2+ rises somewhat locally as a result of this Ca2+ influx.
RyR2 in the sarcoplasmic reticulum membrane senses an increase in intracellular Ca2+ and releases Ca2+ in a physiological reaction that provides positive feedback. Calcium sparks (also known as Ca2+ sparks) are the result of this positive feedback, which is referred to as calcium-induced calcium release.
The cytoplasmic calcium concentration rises throughout the cell as a result of the spatial and temporal summation of around 30,000 Ca2+ spikes. Calcium buffers, which bind a significant amount of intracellular calcium, decrease the rise in cytosolic calcium that occurs after calcium passes through the cell membrane and sarcoplasmic reticulum.
Consequently, a significant rise in total calcium causes a very little increase in free Ca2+.
To free the tropomyosin complex from the actin-binding site and enable the myosin head to attach to the actin filament, the cytoplasmic calcium binds to troponin C.
The contractile mechanism is much the same as that of skeletal muscle (above) from this point on. In short, the myosin head drags the actin filament toward the middle of the sarcomere by hydrolyzing ATP.
Sarco/endoplasmic reticulum ATPase (SERCA) pumps intracellular calcium back into the sarcoplasmic reticulum after systole, preparing the cell for the subsequent cycle. Additionally, the sodium-calcium exchanger (NCX) and, to a lesser extent, a calcium ATPase in the plasma membrane are responsible for the expulsion of calcium from the cell.
The mitochondria also absorb some calcium. Phospholamban is an enzyme that acts as a brake on SERCA. Phospholipase activity is slowed down by phospholamban, which is active at low heart rates, preventing Ca2+ from completely leaving the cell. Phosphorylated and deactivated at high heart rates, phospholamban retrieves most of the Ca2+ from the cytoplasm and returns it to the sarcoplasmic reticulum.
Once more, calcium buffers act as a moderator for this drop in Ca2+ concentration, allowing a significant shift in total calcium to be met with a relatively minor drop in free Ca2+ concentration.
Contractile force is released when the troponin complex separates from the actin filament due to a decrease in Ca2+ concentration. The ventricles can fill with blood and the cardiac cycle can resume when the heart relaxes.
Invertebrate
Circular and longitudinal muscles
Circular and longitudinal muscle cells make up the body wall of annelids like leeches and earthworms, and these cells are what allow the creatures to move. When an earthworm moves through dirt, for instance, its circular and longitudinal muscles contract reciprocally, and the coelomic fluid keeps the earthworm turgid, acting as a hydroskeleton. The anterior part of the animal’s body constricts radially as the circular muscles in the anterior segments contract, pushing the incompressible coelomic fluid forward and lengthening the animal—consequently, the animal’s front end advances.
A wave of longitudinal muscular contractions travels backward when the earthworm’s front end anchors and the circular muscles in its anterior segments relax, pulling the remainder of its following body forward. The term peristalsis refers to these alternating waves of longitudinal and circular contractions that underpin earthworms’ crawling motion.
Obliquely striated muscles
The obliquely striated muscles of invertebrates, such as annelids, mollusks, and nematodes, are made up of bands of thick and thin filaments organized helically instead of transversely, as in the case of the skeletal or cardiac muscles of vertebrates. The obliquely striated muscles of the bivalves can sustain tension for extended periods without using much energy. These muscles help bivalves maintain the closure of their shells.
Asynchronous muscles
The asynchronous muscles that make up the flying muscles of advanced insects including wasps, flies, bees, and beetles. Because of their large and noticeable myofibrils, flying muscles are frequently referred to as fibrillar muscles. These muscles are unique in that they don’t need to be stimulated to contract. Because the quantity of contractions in these muscles does not match, or synchronise, with the quantity of action potentials, they are referred to as asynchronous muscles. For instance, a tethered fly’s wing muscle can beat at a frequency of 120 Hz but can only receive action potentials at 3 Hz.
The muscles are attached to a resonant system that is driven to a naturally occurring vibration frequency, which enables the high-frequency thumping.
Related Testing
Manual Muscle Testing
MMT is used to measure a patient’s muscular strength during a physical examination. It may be applied to assess weakness and is a useful tool for distinguishing between actual weakness and imbalance or low endurance.
The Oxford scale, which doesn’t require any additional equipment and is reliable by independent assessors, is the most regularly used and highly acknowledged technique for measuring muscular strength.
Using the Oxford scale, patients’ strength is measured on a 0–5 scale by testing important muscles in their upper and lower extremities against an examiner’s resistance. The results are recorded as x/5.
- 0: no contraction
- 1: visible/palpable muscle contraction but no movement
- 2: movement with gravity eliminated
- 3: movement against gravity only
- 4: the movement against gravity with some resistance
- 5: movement against gravity with full resistance
Electromyography
The main use of electromyography (EMG) is to differentiate between myopathic and neurogenic muscular weakness and wasting.
EMG is the recording of electrical activity that takes place within a muscle. By identifying the distribution of neurogenic anomalies, EMGs can distinguish localized nerve, plexus, or radicular disease and identify fasciculations or chronic denervation in muscles that seem clinically normal.
A peripheral myopathy’s pathogenesis can be supported by EMGs, which are a necessary inquiry in motor neuron illness to show the broad fasciculation and denervation needed to establish a diagnosis.
A needle electrode is inserted into the muscle to record electromyography readings. A typical EMG needle records a radius of around 1 mm, and there can be 100 muscle fibers in this volume.
Diagnostic data may be obtained by examining the waveforms and firing rates of single- or multiple-motor units.
Pharmacology
From a clinical standpoint, it is critical to understand that a lot of today’s drugs have some effect on muscular contraction.
Therefore, it is essential to comprehend the purposes and ramifications of giving patients medicine, whether it is a side effect or the primary impact of the treatment.
Among the pharmaceutical substances that directly influence muscular contraction include, but are not exclusive to:
Skeletal muscle relaxants (e.g., botulinum toxin, cyclobenzaprine, methocarbamol)
Vasodilating medications (e.g., hydralazine, nitrates, milrinone)
Volatile anesthetics or succinylcholine can lead to malignant hyperthermia
FAQ
The neuromuscular junction, or the synapse between a motoneuron and a muscle fiber, is where skeletal muscle contraction initially starts. Action potentials propagating to the motoneuron and subsequent depolarization cause the presynaptic membrane’s voltage-gated calcium (Ca2+) channels to open.
Actin and myosin, two contractile proteins, combine with calcium to cause muscle contraction, which is a complicated process. As the muscle fibers shorten during contraction, the myosin and actin filaments of the muscle glide past one another.
While stress, physical activity, and dehydration are common reasons, thyroid issues and nerve abnormalities may further raise the risk. Though they can affect any muscle in the body, the calf muscle is frequently affected by these motions. They are rather frequent and often not a reason for alarm.
Muscle spasms, also referred to as cramps, happen when your muscles contract excessively and involuntarily because they are unable to relax. Spasms in the muscles are frequent and natural. They may affect a single muscle, many muscles together, or both.
Muscle contractions can be classified as concentric, isometric, or eccentric. It could be a little deceptive to refer to eccentric contraction as “contraction” because this kind of contraction causes the sarcomere to lengthen.
ATP must be renewed at a pace that is commensurate with ATP demand to maintain muscular contraction. Muscle ATP is restored via three energy systems: mitochondrial respiration, glycolysis, and phosphogen.
eccentric: acting against or in the other direction from a muscle’s contraction. For instance, flexion of the lower arm (bending of the elbow joint) is caused by an outside force, which is controlled by contraction of the triceps and other extensor muscles of the elbow.
ATP, or adenosine triphosphate, is the only substance that powers muscular contraction. The muscle’s ATP reserve will be exhausted at near-maximum hard activity in less than a second, hence ATP has to be continuously synthesized to preserve proper contractile function
Smooth muscle contraction is attributed to the release of calcium via L-type calcium channels or IP3Rs, which are located downstream of Gq-coupled cell-surface receptors. Myosin light-chain (MLC) kinase (MLCK) is stimulated when it binds to calmodulin (CaM). MLC is phosphorylated to promote contraction.
Using the energy from the hydrolysis of ATP, myosin attaches itself to the exposed active site on actin. The actin filament is drawn towards the core as a result. Additionally pulled are the Z lines that are connected to them, causing constriction.